What is bioclimatic architecture?
Buildings are among the world’s largest energy consumers!
Residential and commercial buildings account for over 60% of global electricity consumption.
Forecasts indicate that between 2007 and 2035, the use of oil, coal, and natural gas may increase by 30–50%, potentially leading to a drastic rise in CO₂ emissions by 2050.
This trend further intensifies the effects of climate change, making it increasingly urgent to reduce energy consumption and adopt environmentally conscious architectural solutions.
Bioclimatic architecture is an approach that designs buildings based on local climate conditions and natural resources.
The goal is to ensure that the building maximizes the use of natural energy sources—such as sunlight, ventilation, and thermal mass—to minimize its overall energy demand.
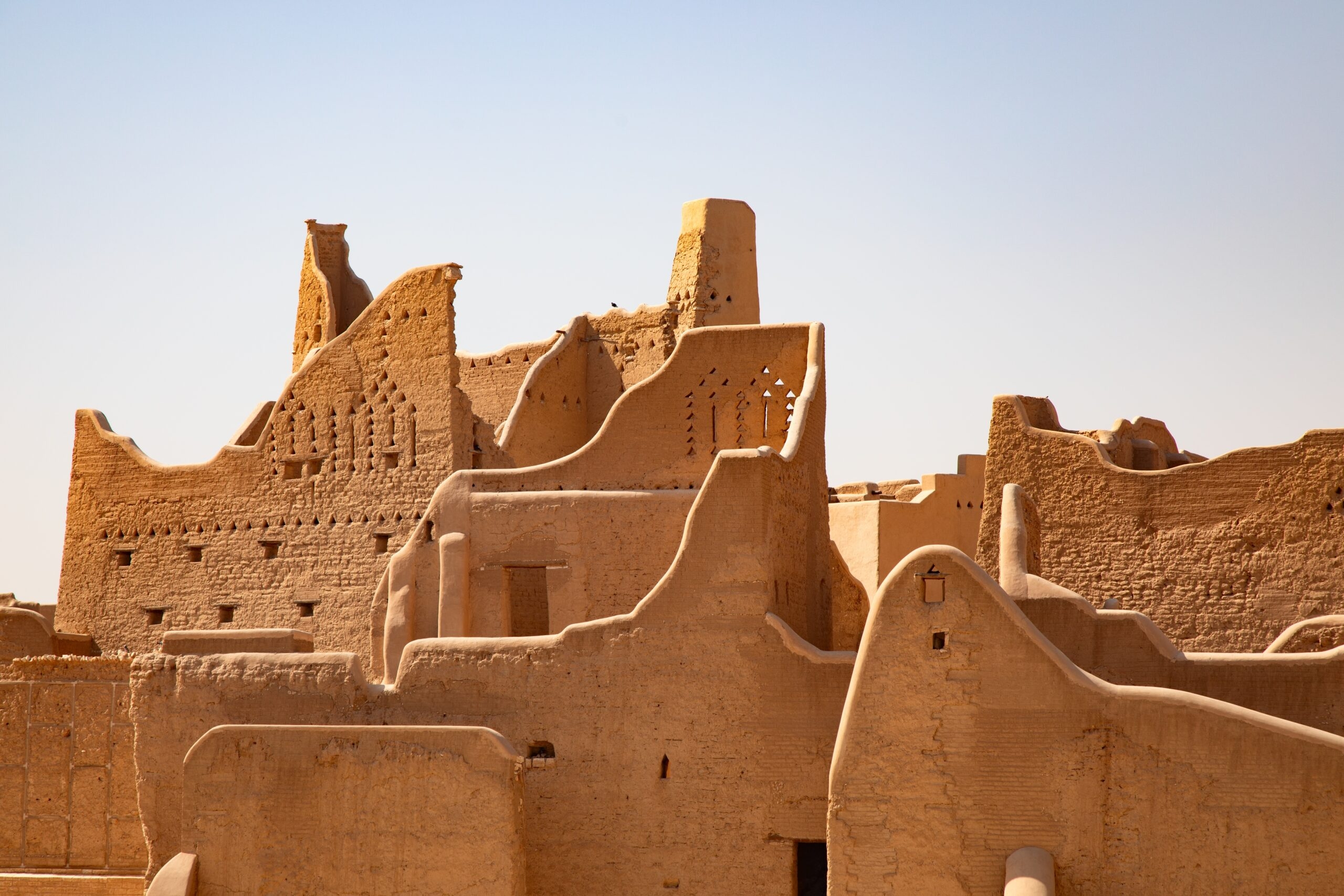
The evolution of bioclimatic architecture
Traditional architectural forms and techniques evolved over centuries in direct response to local climate conditions, with buildings relying on passive strategies to regulate indoor temperature and ensure thermal comfort.
There are numerous examples from different parts of the world that illustrate this climate-responsive approach:
- Mediterranean houses with thick stone walls that keep interiors cool in summer and warm in winter.
- Wind catchers (badgir) in the Arab world, which provide natural ventilation in hot, arid climates.
- Timber houses in Japan and Scandinavia, known for their excellent thermal insulation suited to cold environments.
However, following the Industrial Revolution, the widespread use of new construction materials—such as concrete, steel, and glass—along with the easy availability of energy resources fundamentally transformed architecture.
By the 1950s and 1960s, with the rise of modernist architecture, glass façades, air conditioning systems, and mechanical services increasingly replaced traditional, climate-responsive building techniques.
Building orientation and natural thermal regulation were often neglected, as the availability of artificial heating and cooling systems seemed to liberate buildings from their environmental context.
This new approach was built on the assumption of cheap energy—and largely ignored the long-term sustainability of natural resources.
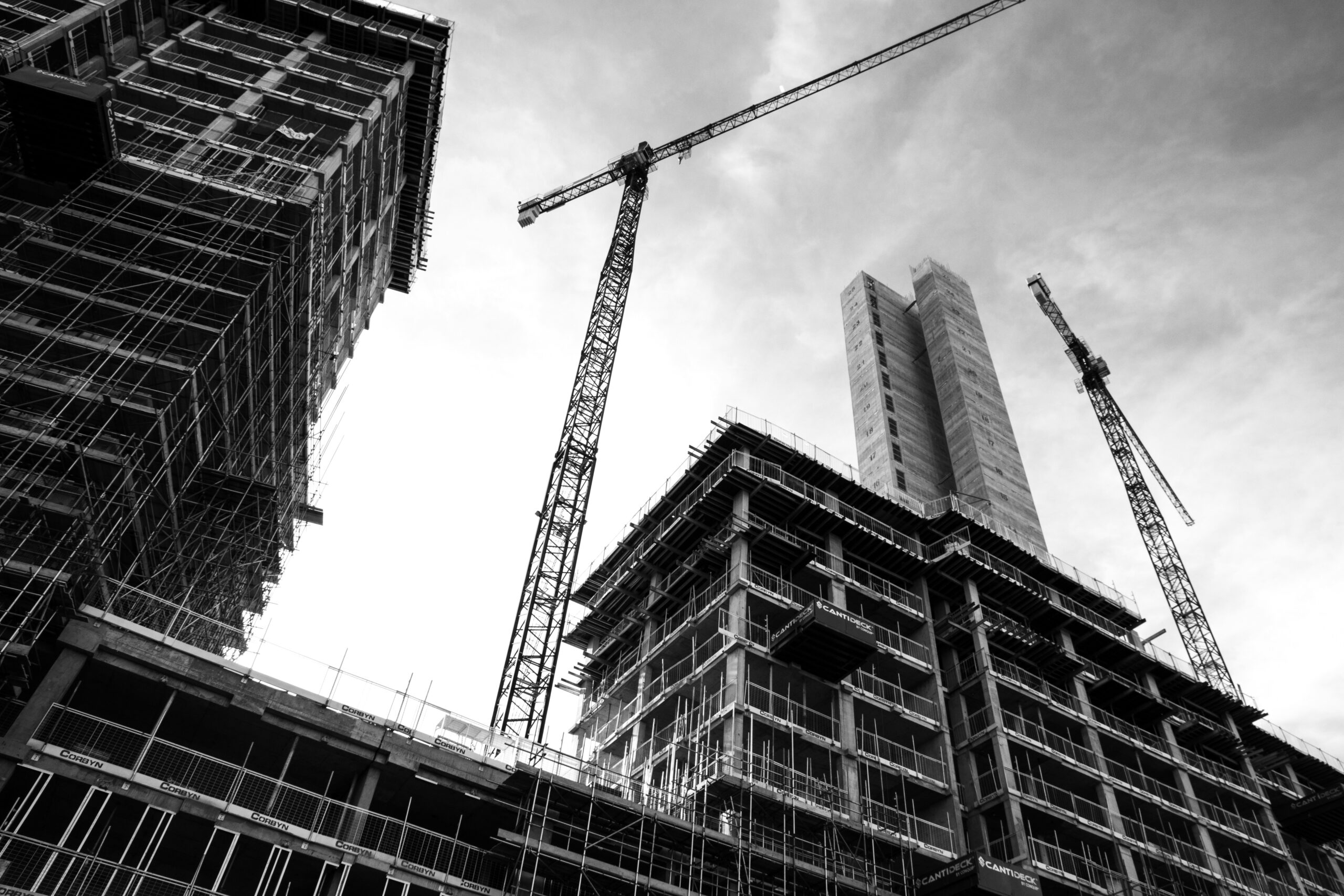
Climate-responsive design
Climate-responsive design is a more advanced and intentional evolution of bioclimatic architecture, incorporating not only traditional knowledge but also modern materials and technologies.
It blends time-tested, experience-based methods with scientific data and climate simulations to minimize a building’s energy use—without compromising occupant comfort.
As early as the 1920s and 1930s, modern architecture had already begun to recognize the importance of sunlight and building orientation—reflected in the work of renowned architects such as Le Corbusier and Alvar Aalto.
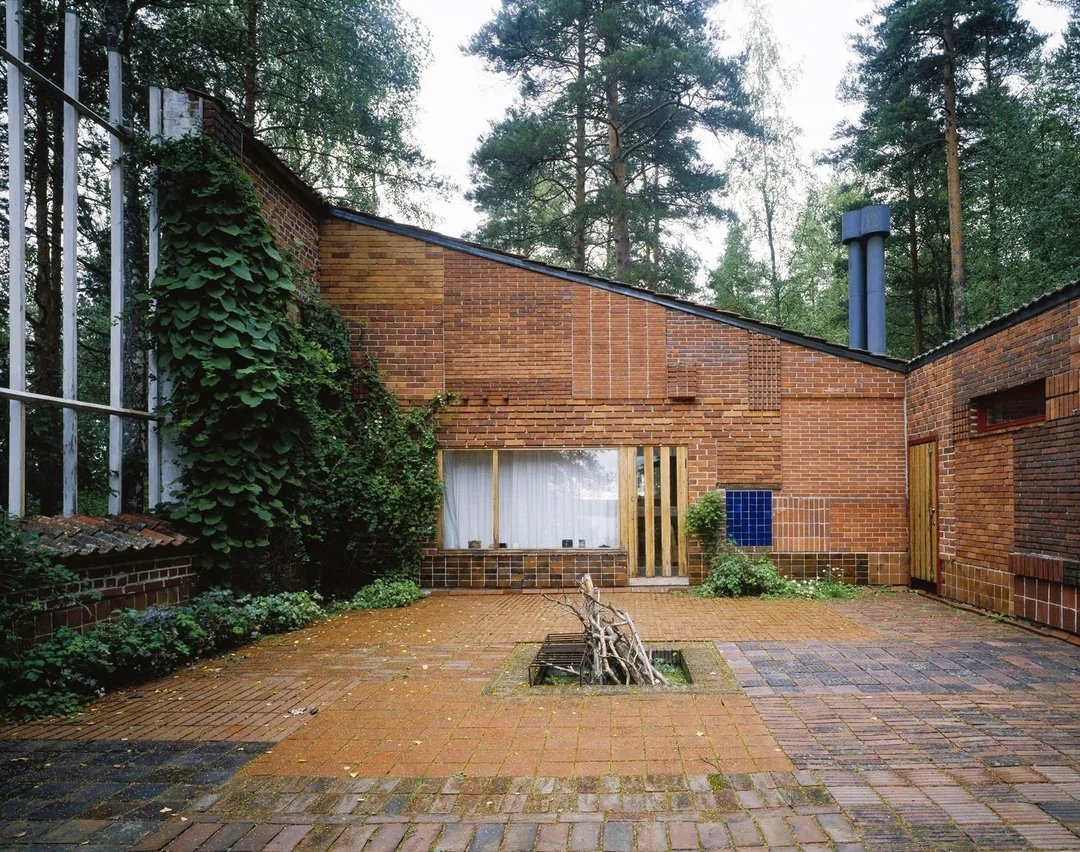
By the 1940s and 1950s, architectural design was increasingly informed by scientific approaches that aimed to improve buildings’ responsiveness to climate.
In the 1950s, the Form and Climate research group at Columbia University developed some of the first design techniques focused on climate adaptation, while the American Institute of Architects (AIA) published climate analysis charts to support the environmental assessment of U.S. cities.
A major breakthrough came with the work of Victor and Aladar Olgyay, who introduced the bioclimatic chart—a key design tool that helped architects create energy-efficient buildings tailored to their climate (Pontes et al., 2022).
Throughout the 1970s and 1980s, climate-responsive architecture continued to evolve rapidly, propelled by the rise of computer-aided design (CAD) and an increasing emphasis on energy performance research.
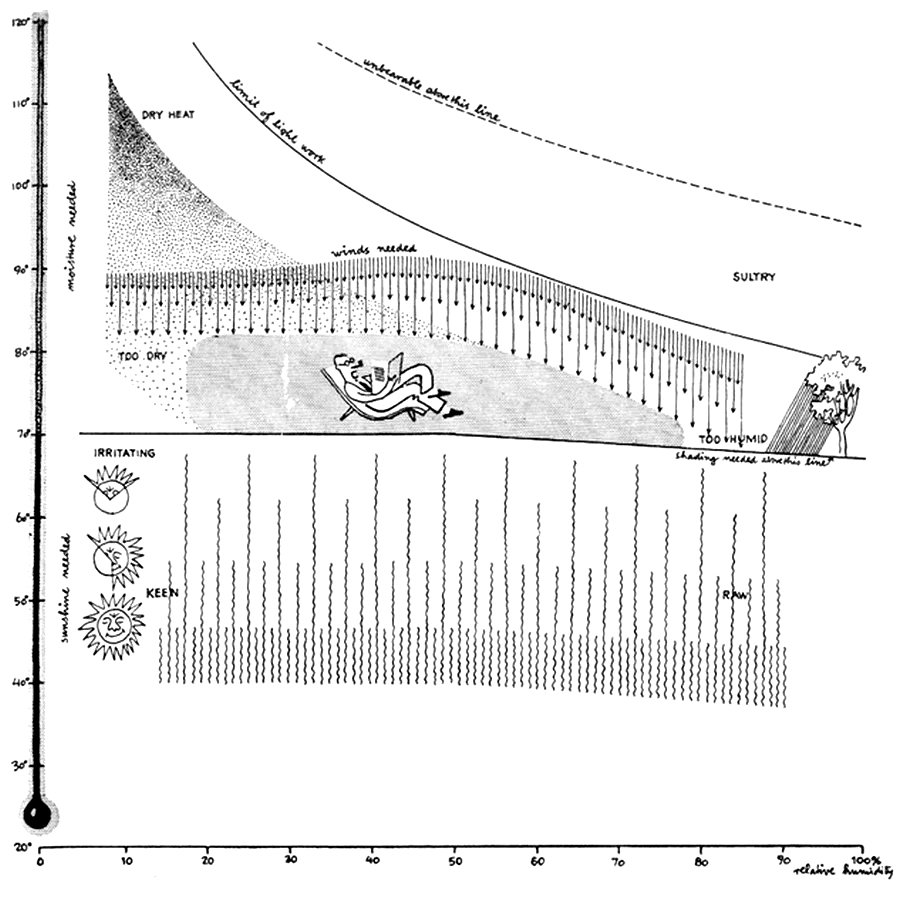
Climate analysis and modeling
Climate analysis and modeling aim to improve the energy efficiency, comfort, and resilience of buildings and urban environments by using detailed environmental data.
This approach helps optimize a building’s orientation, massing, and functional layout based on key local climate factors—such as temperature, solar exposure, wind patterns, humidity, and topography.
The process typically involves two main stages:
- Climate analysis – collecting and interpreting site-specific climate data
- Modeling – running simulations to predict how environmental conditions will affect building performance
From the 1970s onward, tools like Murray Milne’s Climate Consultant and the standardized EnergyPlus Weather (EPW) file format paved the way for climate-responsive design.
In the early 2000s, Andrew Marsh introduced Ecotect and Weather Tool, which allowed architects to integrate climate data directly into the digital design workflow.
Today, advanced platforms like Ladybug Tools, Climate Studio, and Cove.tool enable intuitive, visual climate analysis within leading design environments such as Autodesk Revit and Rhinoceros 3D.
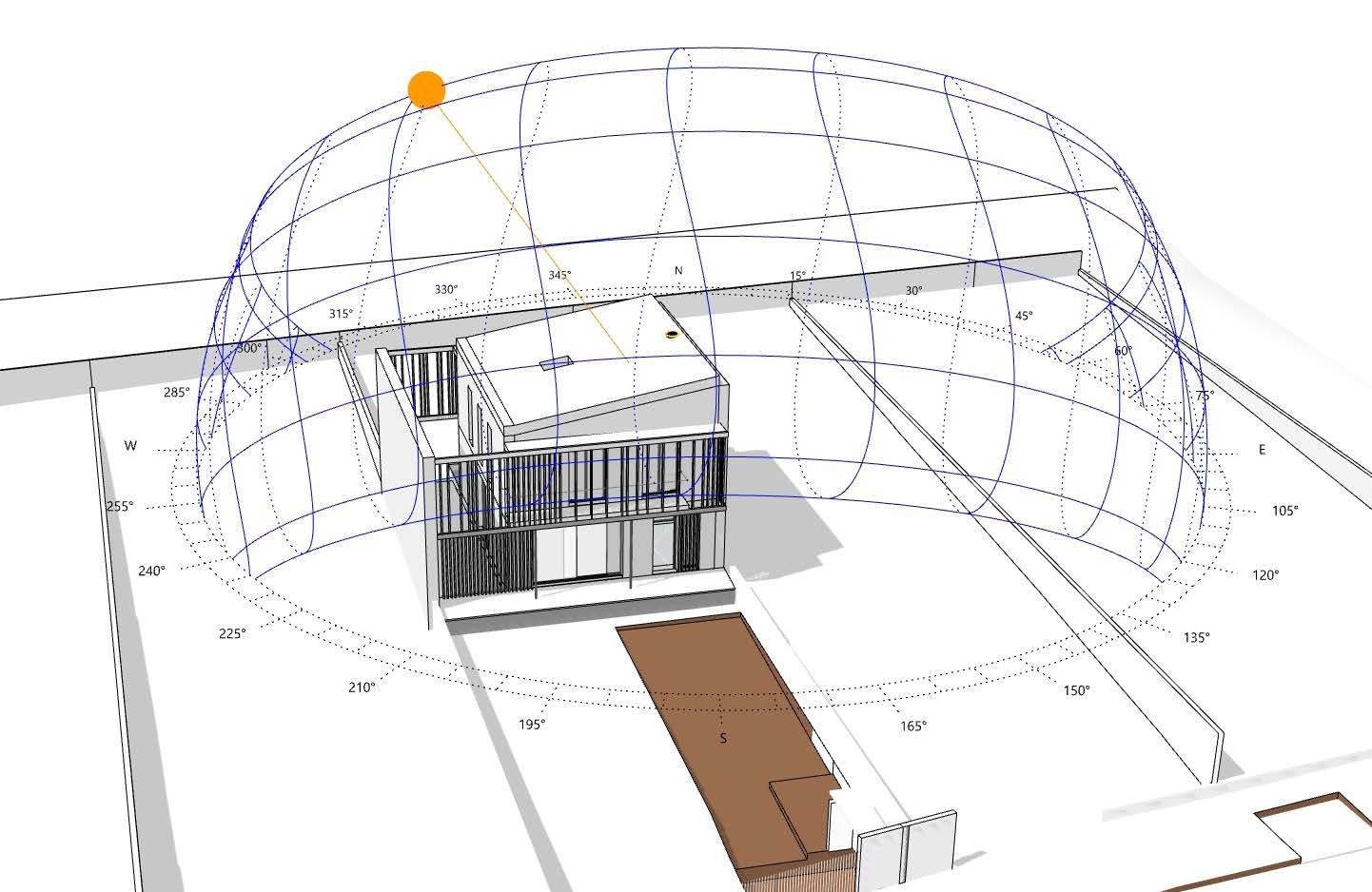
In summary:
At Equinox, climate-responsive design is not just a theory—it’s embedded in how we design.
From the very start of each project, we integrate passive strategies such as natural ventilation, solar heat gain, and shading into the core of our decision-making process.
This holistic mindset not only helps lower operational costs and improve user comfort, but also enhances the resilience of buildings—ensuring they remain adaptable and efficient in the face of a changing climate.
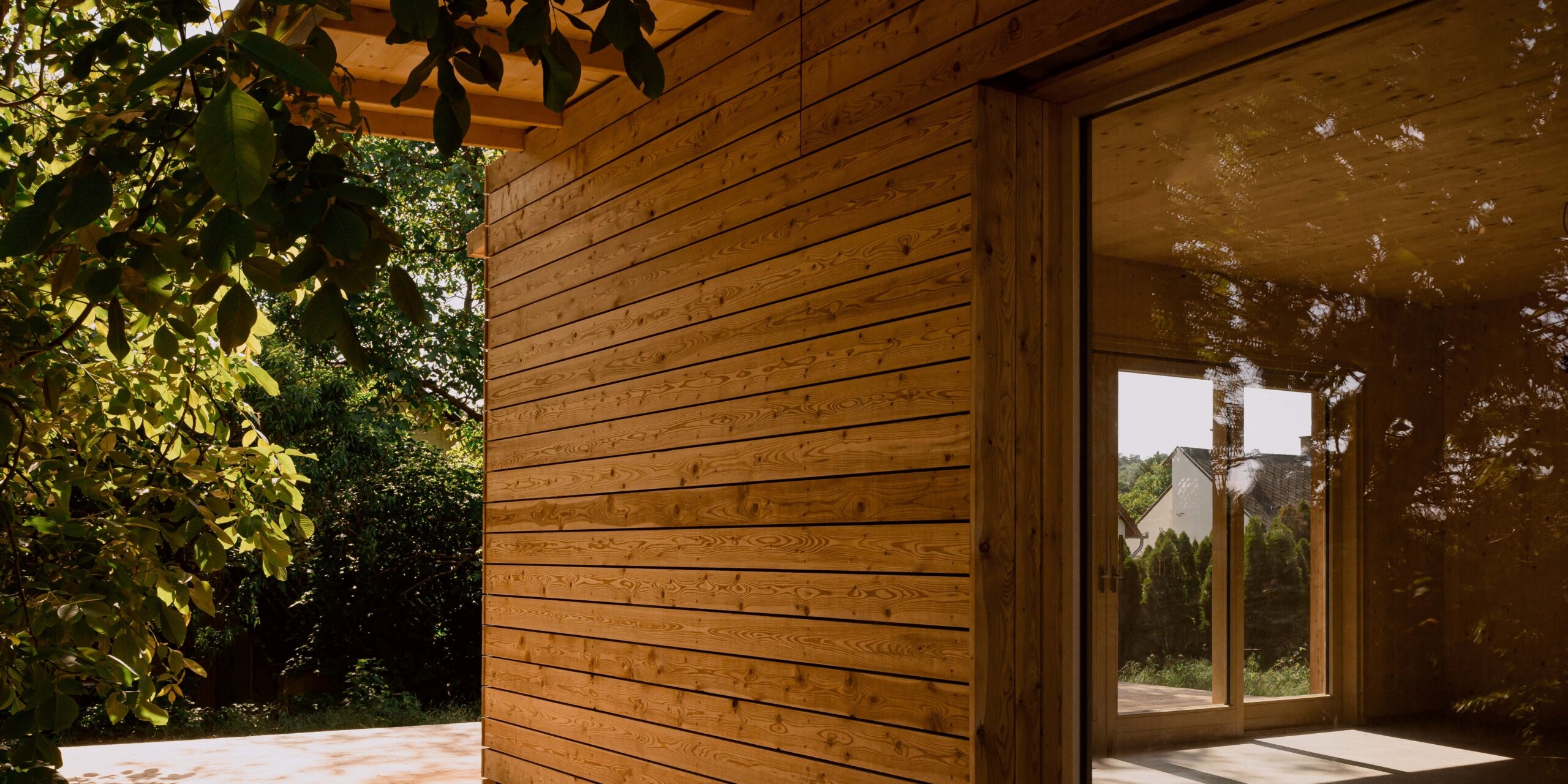
Climate-responsive building design
At Equinox, every design process begins with modeling the local climatic conditions. Whether it’s a new build or a renovation, this is the foundation that informs every design decision that follows.
Climate data & adaptive comfort
Using real meteorological data—sun path, wind direction, humidity, and temperature—we carry out an adaptive comfort analysis tailored to the project location.
This means we don’t just look at temperature values. We evaluate how people actually experience comfort, factoring in local climate conditions, lifestyle, and cultural expectations.
By grounding our work in real-world data, not idealized assumptions, we ensure that each building is designed to perform comfortably and efficiently—in harmony with its environment.
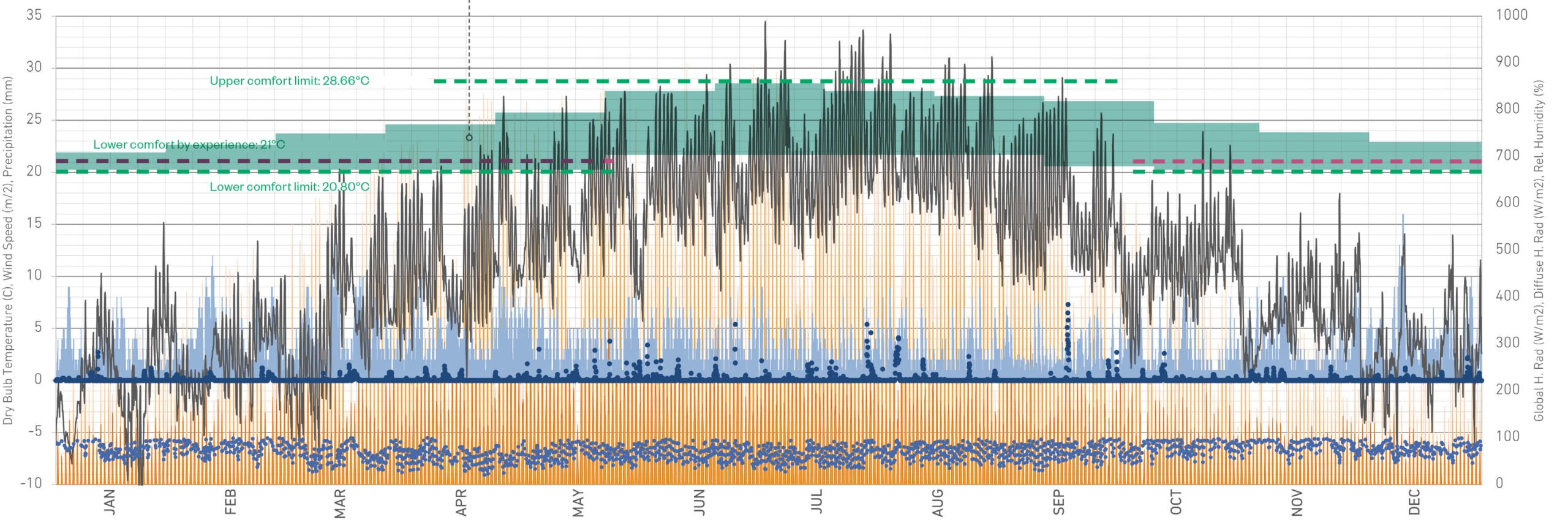
Sensitivity analysis
A key step in the climate-responsive design process is conducting a sensitivity analysis, performed after placing the proposed building within a simulated climatic environment based on real meteorological data (e.g. from an EPW file).
During this process, we systematically modify selected architectural and technical parameters to identify:
- Which factors have the greatest impact on indoor thermal comfort, natural daylighting, and ventilation performance?
- Which design interventions most effectively reduce annual energy demand (heating, cooling, lighting)?
Typical variables examined include:
- Building orientation and massing,
- Glazing ratio, placement, and orientation,
- Effectiveness of passive shading strategies (e.g. overhangs, louvers, shutters),
- Thermal inertia and material composition of façade and roof assemblies.
The results are delivered both numerically and visually, providing the design team with actionable feedback on:
- Potential overheating zones,
- Shading requirements throughout the year,
- Daylight availability in interior spaces, and
- Annual energy consumption across different design iterations.
This methodology aligns with international standards and comfort criteria, including CIBSE TM52, EN 16798, and DIN 4108-2, supporting informed, performance-based design decisions.
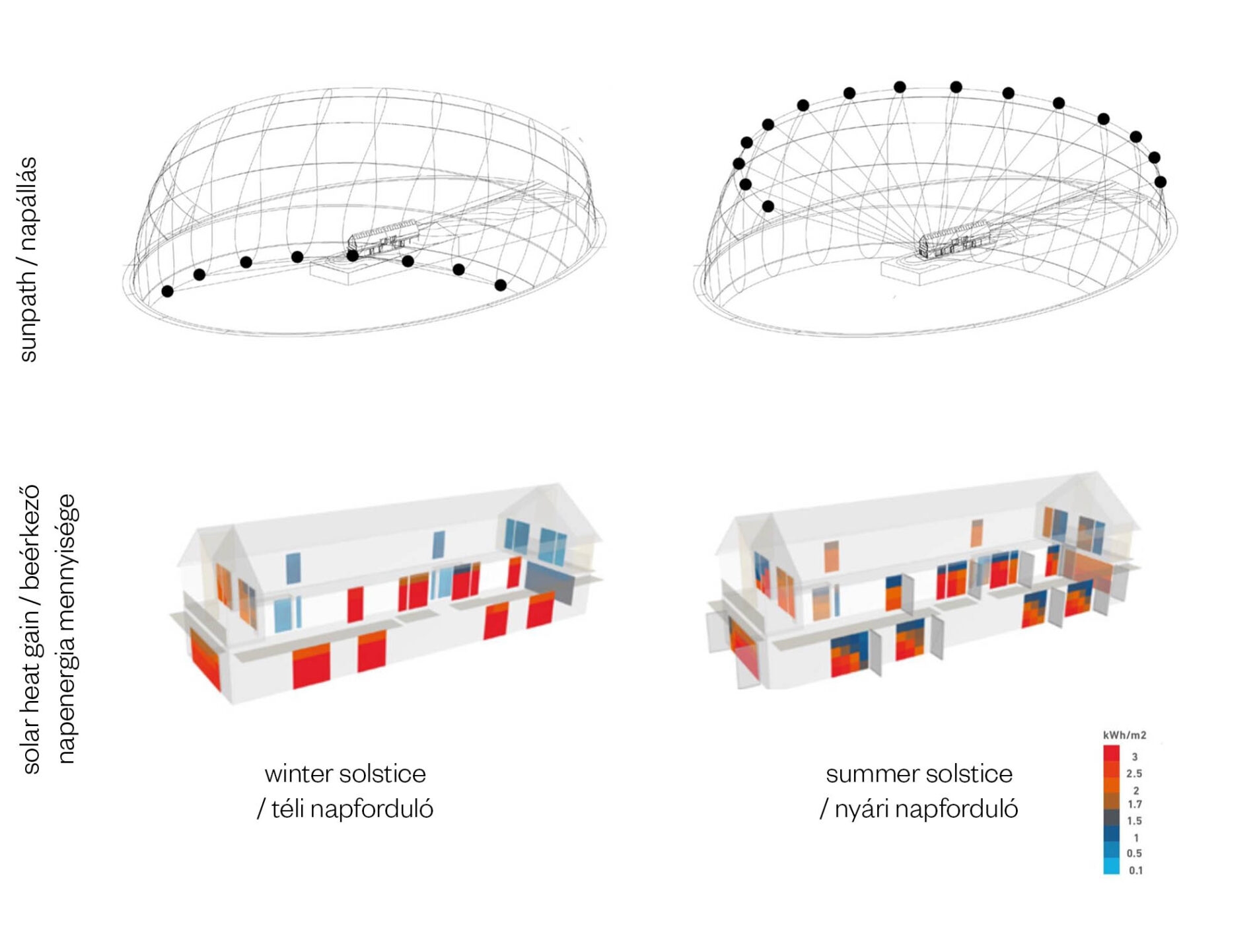
Daylight analysis
As part of our design process, we carry out a daylight analysis to assess how much natural light reaches each level of the building under the proposed design conditions.
This analysis is guided by EN 17037, the first comprehensive European standard (introduced in 2018) dedicated to daylight in indoor spaces.
Unlike older methods, EN 17037 considers not only the amount of daylight but also its quality, including:
- Access to outside views
- Even distribution of light across the interior
- Duration of sunlight exposure throughout the year
- Visual comfort for occupants.
A key metric we use is sDA – Spatial Daylight Autonomy, which evaluates whether:
- At least 50% of the occupied floor area
- Receives a minimum of 300 lux of natural light
- During at least 50% of the annual occupied hours (typically between 8:00 and 18:00)
- Without relying on artificial lighting
We typically aim to meet or exceed the sDA 300/50% threshold, ensuring high levels of daylight performance, visual comfort, and energy efficiency across the design.
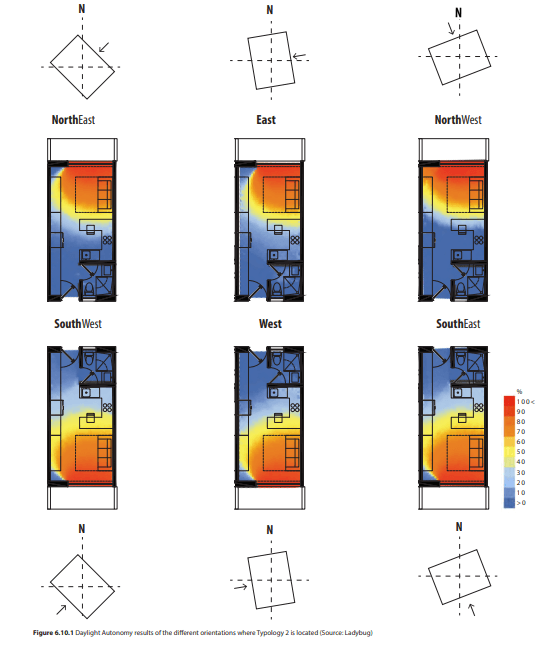
Solar heat gain analysis
As part of our energy efficiency strategy, we evaluate solar heat gain—the amount of heat energy from sunlight that enters a building and affects its internal temperature.
This involves a solar radiation analysis, which helps us understand how much sunlight the building receives during warmer periods and how that impacts indoor heat loads.
We use this data to inform key design decisions, including:
- Shading system design and sizing
- Façade and window layout
- Development of passive and active cooling strategies
- Thermal comfort and overheating assessments, in line with EN 16798 and EN 17037
We also assess internal heat gains—the heat generated within the building from various sources, which can significantly influence cooling and heating needs.
Typical sources of internal heat gain include:
- People (body heat)
- Lighting systems
- Electrical appliances and equipment
- Sunlight entering through windows and warming interior surfaces.
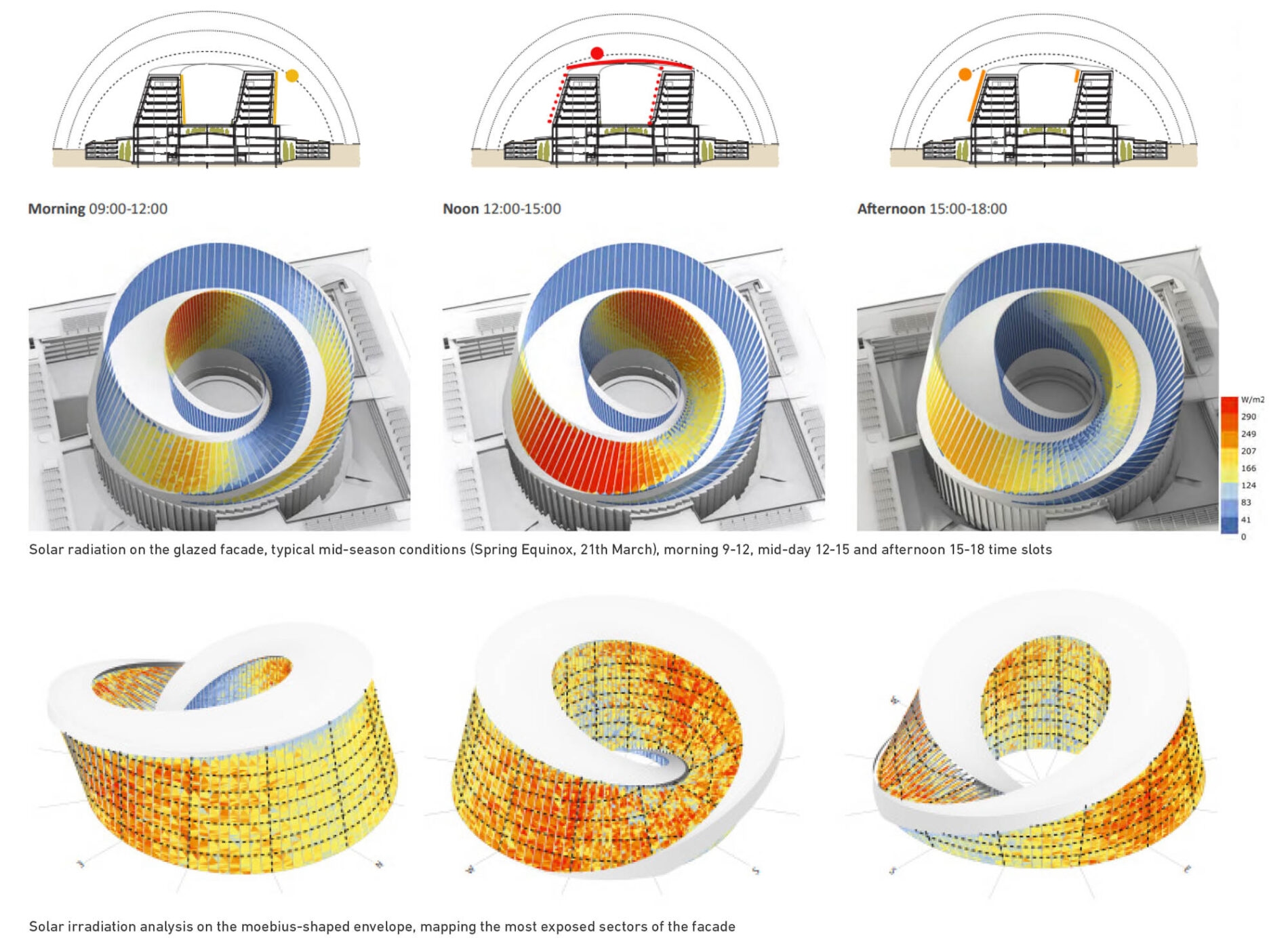
Wind analysis
Understanding prevailing wind patterns is a key part of climate-responsive building design, as wind plays a crucial role in natural ventilation, thermal comfort, and energy performance.
What do we assess?
- We analyze meteorological data, focusing on annual and seasonal wind direction trends.
- This data comes from official weather stations (such as OMSZ in Hungary) or from EPW-format weather files (EnergyPlus Weather).
Using this data, we generate wind rose diagrams to visualize how frequently and how strongly wind blows from different directions throughout the year.
This analysis directly informs the architectural layout and form of the building, particularly the placement of openings and outdoor spaces:
1. Natural ventilation Windows and openings are strategically positioned to take advantage of prevailing breezes, enabling cross-ventilation between key zones like living and sleeping areas.
2. Wind protection In colder or harsher climates, façades facing dominant winds are designed with fewer or smaller openings to minimize heat loss and improve occupant comfort.
3. Shelter for outdoor areas Courtyards, terraces, and entrances are located in wind-protected zones, making them more usable throughout the year.
4. Energy efficiency By directing airflow effectively, buildings can stay cooler in summer without relying on mechanical cooling systems—reducing energy demand and improving sustainability.
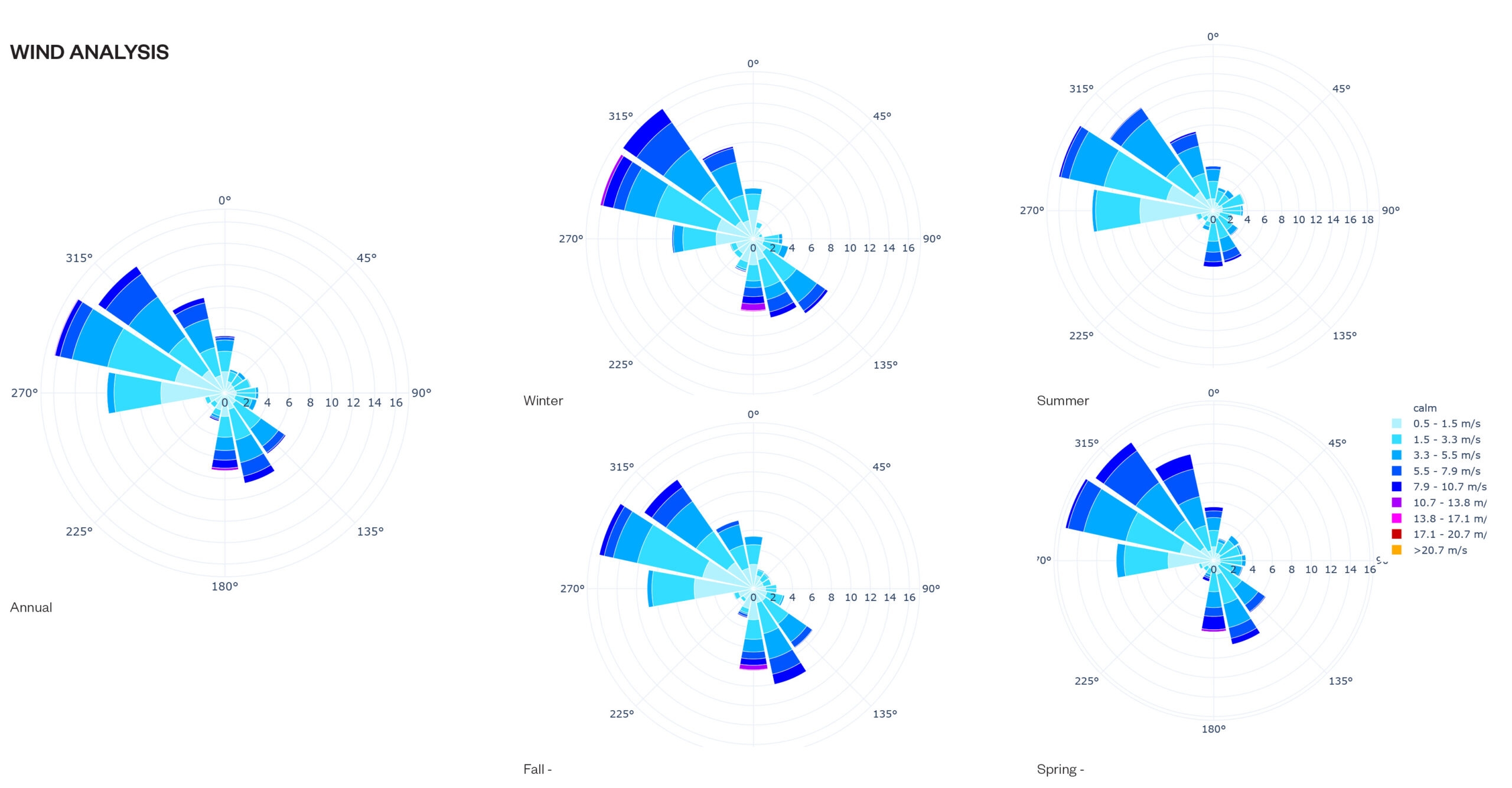
Natural ventilation
The CIBSE AM10 and TM37 guidelines provide key principles for designing natural ventilation strategies, with a focus on thermal comfort, indoor air quality, and energy efficiency.
Main types of natural ventilation:
- Cross ventilation – wind-driven air flows through openings on opposite sides of a space
- Stack ventilation – warm air rises and exits at high level, drawing in cooler air from below
- Hybrid (mixed-mode) systems – a combination of natural and mechanical ventilation
These guides outline methods for predicting airflow, indoor temperatures, and ventilation effectiveness.
The goal is to reduce dependence on mechanical systems and support the creation of energy-efficient, sustainable buildings.
During the design process, we analyze airflow potential across different building sections.
Based on these studies, we determine the optimal placement and operation of windows and openings to maximize passive ventilation performance.
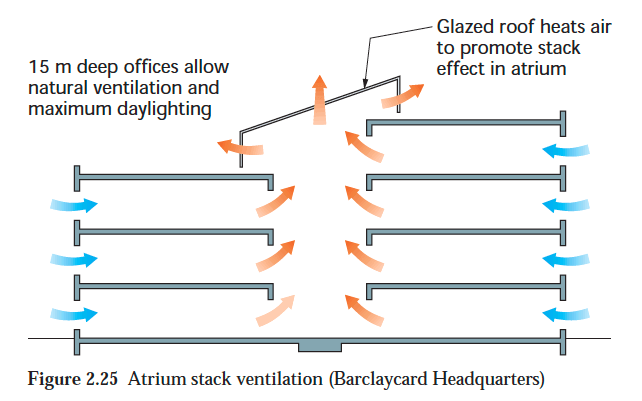
The long-term value of climate analysis
Energy efficiency and sustainability
- With proper orientation and passive thermal design strategies, a building’s energy consumption can be reduced by 50–80%, according to the International Energy Agency.
- The Passive House standard, developed in Germany, can reduce heating energy demand by up to 90% compared to conventional buildings.
Comfort and health
Natural daylight and ventilation can reduce indoor air pollutants by up to 30%, improving occupant health and productivity (Harvard T.H. Chan School of Public Health).
Economic benefits
Sustainable buildings may have up to 20% higher property value compared to conventional buildings, according to the World Green Building Council.
Reduced environmental impact
Sustainable buildings emit 30–40% less CO₂ during operation, significantly lowering their environmental footprint.
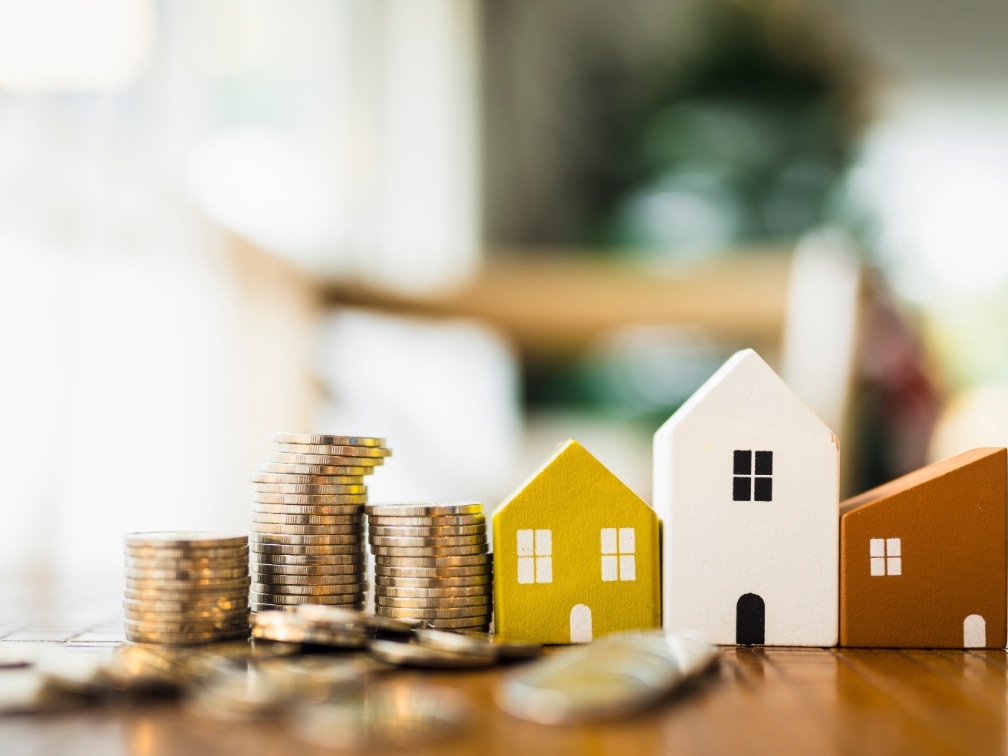
Key supplementary factors
- Considering local climate conditions is essential, as not all sustainable technologies perform equally well across different regions.
- Modern systems and materials also require expert installation and regular maintenance—without this, their performance and efficiency can quickly decline.
- Beyond construction and operational costs, it is crucial to conduct a full life-cycle environmental impact assessment to ensure that solutions are genuinely sustainable.
- User education is equally vital—sustainable solutions can only reach their full potential when occupants understand how to operate buildings efficiently.
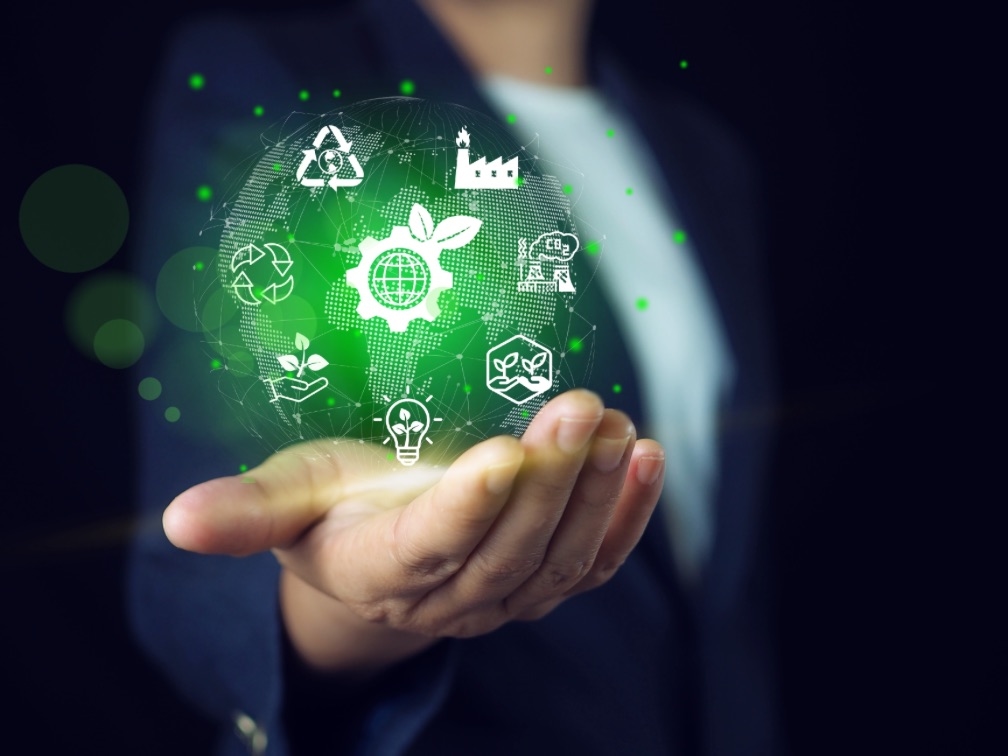
How can equinox support your organization?
At Equinox, we believe that a well-designed building should live in harmony with its environment.
We combine bioclimatic design principles, digital modeling, and sustainable materials to create homes and buildings that naturally adapt to the local climate, consume less energy, and offer healthy, comfortable living spaces.
Whether you're planning a Passive House or a climate-conscious renovation, we guide you through every step — from the first concept to completion.
Our experienced team is here to help you find the most energy-efficient and environmentally friendly solutions tailored to your needs.
Request a proposal
To ensure a tailored and precise proposal for your project, please provide the following details:
- Type of project (residential, office, public building, etc.)
- Site location and climatic conditions
- Sustainability objectives (e.g., net-zero energy, renewable energy integration)
- Current project stage (concept design, permitting, construction, etc.)
- Budget considerations and operational requirements
Get in touch with us!
Cited and additional literature
Policies
- 2022 Global Status Report for Buildings and Construction. United Nations Environment Programme (2022).
- Energy in Buildings and Communities Programme (EBC). International Energy Agency (IEA) (2023).
- Directive 2010/31/EU on the Energy Performance of Buildings (Nearly Zero-Energy Buildings - NZEB). European Commission (2010). Official Journal of the European Union.
- Energy Performance of Buildings Directive (EPBD). European Commission (2024).
Books
- Victor Olgyay & Aladar Olgyay: Design with Climate: Bioclimatic Approach to Architectural Regionalism. Princeton University Press (1963).
- Lányi Erzsébet: Sustainable Built Environment: Principles and Architectural Tools for a Paradigm Shift (PhD dissertation, 2010). Budapest University of Technology and Economics.
- Richard Hyde: Climate Responsive Design: A Study of Buildings in Moderate and Hot Humid Climates. E & FN Spon (2000).
Articles
- Coch, H. (1998). Bioclimatic design: A solution to climate change. Renewable and Sustainable Energy Reviews, 2(1-2), 67–85.
- Pontes, R. H., Najjar, M. K., Hammad, A. W. A., Vazquez, E., & Haddad, A. (2022). Adapting the Olgyay bioclimatic chart to assess local thermal comfort levels in urban regions. Clean Technologies and Environmental Policy, 24(2), 661–675.
- World Green Building Council. (2021). The business case for green buildings.